Adsorption of Amoxicillin using Oxidized Carbon Nanotubes of Aqueous Solution
Alireza Falahati Marvast1, Ali Khanzadeh Poshtiri2
, Farnaz Doniamali3, Alireza Saegh4, Davoud Balarak5*
and Shaziya Haseeb Siddiqui6
1Department of Toxicology and Pharmacology, School of Pharmacy and Pharmaceutical Sciences, Shahid Sadoughi University of Medical Sciences, Yazd, Iran.
2Department of Natural Science, Gorgan University of agriculture and natural science, Gorgan, Iran
3Department chemistry, PNU University Qazvin, Qazvin, Iran.
4Department of Pharmacy, Shahid Sadoughi University of Medical Sciences, Yazd, Iran.
5Department of Environmental Health, Health Promotion Research Center, Zahedan University of Medical Sciences, Zahedan, Iran
6Department of Chemistry, Sam Higginbottom University of Agriculture Technology and Sciences (SHUATS), Allahabad, Uttar Pradesh, India.
Corresponding Author E-mail:dbalarak2@gmail.com
DOI : http://dx.doi.org/10.13005/ojc/400504
Article Received on : 05 Aug 2024
Article Accepted on : 30 Sep 2024
Article Published : 05 Nov 2024
Reviewed by: Dr. Hasan Tahermansuri
Second Review by: Dr. Voltaire Organo
Final Approval by: Dr. Ioana Stanciu
The extensive therapeutic repertoire of antibiotics for both humans and animals has resulted in the direct or indirect release of these compounds into the environment, particularly into water ecosystems. Traditional methods for eliminating antibiotic residue from wastewater have proven to be largely ineffective, leading to a need for alternative treatments. As a result, there has been a significant increase in the attention given to other methods of antibiotic residue elimination. This paper presents the kinetic adsorption of Amoxicillin (AMX) onto Oxidized multiwalled Carbon Nanotubes (OMWCNTs). In the batch adsorption experiments, the focus was on exploring how initial concentration, temperature variations, and contact time affect the percentage of removal. Optimization strategies were then implemented to maximize the AMX adsorption capacity concurrently. A maximum of 98.71% AMX was removed at an optimum contact time 75 min and temperature 40 The rise in temperature led to an increase in adsorption capacity, signifying the endothermic nature of the adsorption reaction of AMX onto OMWCNTs, as observed in this study. When compared with other kinetic models, the R2 obtained using the PSO rate equation are markedly higher. Mechanisms such as electrostatic interactions, hydrophobic interactions, hydrogen bonding, and others facilitate antibiotic removal by OMWCNTs, providing technical backing for antibiotic wastewater treatment.
KEYWORDS:Adsorption; Amoxicillin; Kinetics; Oxidized Carbon Nanotubes; Thermodynamics
Download this article as:
Copy the following to cite this article: Marvast A. F, Poshtiri A. K, Doniamali F, Saegh A, Balarak D, Siddiqui S. H. Adsorption of Amoxicillin using Oxidized Carbon Nanotubes of Aqueous Solution. Orient J Chem 2024;40(5). |
Copy the following to cite this URL: Marvast A. F, Poshtiri A. K, Doniamali F, Saegh A, Balarak D, Siddiqui S. H. Adsorption of Amoxicillin using Oxidized Carbon Nanotubes of Aqueous Solution. Orient J Chem 2024;40(5). Available from: https://bit.ly/4efhsd6 |
Introduction
The widespread use of antibiotics has become a major concern due to their negative effects on both health and the environment 1, 2. Studies have shown the detection of antibiotics in various aquatic environments and water supplies. In most cases, these antibiotics exist as mixtures rather than individual compounds 3, 4. Even at low levels, the persistence of antibiotics in water bodies can promote the emergence of antibiotic-resistant genes and harm aquatic organisms, leading to ecosystem disruption and potential threats to human health 5. Therefore, the development of efficient methods for the removal of antibiotics from aquatic environments is imperative 6, 7.
A variety of techniques have recently been explored for wastewater treatment, including biodegradation, adsorption, ion exchange, membrane separation methods, and catalytic oxidation, all aimed at degrading and eliminating antibiotics 8, 9. Among these techniques, adsorption processes offer a promising approach for eliminating antibiotics due to their efficiency, simplicity, flexibility, and cost-effectiveness 10. Effective water purification and antibiotic removal require adsorbents with high adsorption capacity and specific selectivity, attributes typically found in porous adsorbents with large pore diameters and abundant functional groups such as -OH and -COOH 11, 12. However, the adsorption of large-molecule antibiotics from aqueous solutions by adsorbents with smaller pore sizes is hindered by the size exclusion effect. Additionally, the presence of numerous functional groups on material surfaces enhances antibiotic removal from wastewater 13, 14. Mesoporous carbon, with its and abundant functional groups, high surface area, and large pore volume, emerges as a promising candidate for adsorbing large-molecule contaminants 15, 16.
The limited biodegradability of antibiotics renders conventional biological treatment processes relatively ineffective in treating antibiotic manufacturing wastewater 17, 18. Consequently, physical or chemical treatments are often favored. Nonetheless, these methods can be costly and may not adequately address the broad spectrum of antibiotic wastewaters 19, 20. AC serves as a popular adsorbent for antibiotic removal through adsorption 21. Nevertheless, the expensive nature of AC has driven the exploration and adoption of numerous alternative adsorbents for removing antibiotics from aqueous solutions 22, 23.
Ever since their discovery, CNTs have been extensively studied across various disciplines 24. The vast potential for future engineering applications is indicated by the exceptional mechanical properties, remarkable electrical conductivity, small size, and large surface area of these materials 25, 26. Within the spectrum of CNTs, SWCNTs and MWCNTs are delineated based on the number of layers they exhibit 27. The production approaches for MWCNTs are relatively straightforward and can be easily scaled up to accommodate large-scale production.
Carbon nanotubes (CNTs) are cylindrical structures composed of one or more rolled-up sheets of graphene. Oxidation refers to the process of introducing oxygen-containing functional groups (like carboxylic acid, hydroxyl, and carbonyl) onto the surface of CNTs. This chemical modification significantly alters their physical and chemical properties 20.
Pristine CNTs tend to aggregate due to strong van der Waals forces. Oxidation introduces polar groups, enhancing their solubility in polar solvents and improving dispersion, making them easier to process and integrate into composites. Functional groups introduced through oxidation increase CNT reactivity, enabling better adhesion to other materials and facilitating chemical modification for specific applications 21. Oxidized CNTs exhibit increased wettability, making them suitable for applications involving contact with liquids, such as filtration and sensors. In some cases, oxidation can enhance the electrical conductivity of CNTs by creating defects that act as charge carriers 22. In this study, we used oxidized carbon nanotubes to remove amoxicillin, which has not been used in other studies.
Materials and Methods
The main chemical employed in our study, as the pollutant, was AMX prepared by Merck (Darmstadt, Germany). Fig. 1 illustrates the chemical structure of AMX. To adjust the pH of the solution, either 0.1 M H2SO4 or NaOH was used. Experiments were accomplished in conical flasks (250 mL), in batch mode.
![]() |
Figure 1: Chemical structure of the used AMX |
For the production of MWCNTs, chemical vapor deposition (CVD) of acetylene in a hydrogen atmosphere at 760 ◦C was employed, with Ni–Fe nanoparticles serving as catalysts. Fe(NO3)2 and Ni(NO3)2 underwent a sol–gel process and subsequent calcination to obtain FeO and NiO, respectively. Fe and Ni nanoparticles were generated through deoxidation of the mentioned compounds using H2. The ends of the tubes were uncapped by placing the raw MWCNTs in a liquid consisting of 3M HNO3. We did this so we can use the MWCNTs for something else. We mixed 3 grams of MWCNTs with 400 mL of 3 M HNO3 and used sound waves to stir it for 24 hours. After that, we filtered the mixture and rinsed it with clean water until the liquid had a pH of about 6. Then, we dried the mixture at 80 degrees Celsius. Following this, the oxidized MWCNTs underwent a calcination process at 450 ◦C for 24 hours to eliminate any remaining amorphous carbon, after which they were utilized in the subsequent experiments. The experiments used chemicals that were of analytical purity and were directly employed without any additional purification steps. Additionally, Milli-Q water was utilized to prepare the solutions.
The experiments were fulfilled at ambient conditions and T = 28 ± 2 ◦C; for this purpose, polyethylene tubes, as batch system, were employed. To conduct the measurements, a specific quantity of OMWCNT was mixed with AMX solution (this was done in 100 mL flasks) and shaken at a fixed rate of 200 rpm for a certain period. Once the adsorption experiments were finished, an external magnet was used to separate the OMWCNT. UV-vis spectroscopy was used to track changes in absorbance of AMX solution samples at specific time intervals. The maximum wavelengths (λmax) of 622 nm were utilized for the determination of the changes. The removal ratio (R%) of AMX was computed by comparing its initial and equilibrium concentrations. All the experimental results were obtained from duplicate determinations and had a relative error of roughly 5%.
The thermodynamic parameters, including the free energy change (ΔG0), enthalpy change (ΔH0), and entropy change (ΔS0), were estimated using the equations presented below 20.
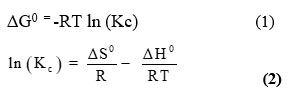
Kc is the symbol used to represent the sorption equilibrium constant, R is the symbol used to represent the gas constant, and T is the symbol used to indicate the absolute temperature in Kelvin.
Results and Discussion
Analysis using the N2-BET method revealed the specific surface area of the OMWCNTs to be 214 m2/g. Subsequent examination of the OMWCNT morphologies was conducted via scanning electron microscopy (SEM) utilizing a JEOL JSM-6700F instrument (see Fig. 2). The SEM illustrates OMWCNTs characterized by exceptionally smooth surfaces, with diameters varying from 10 to 30 nm and lengths measuring several micrometers.
![]() |
Figure 2: SEM image of OMWCNTs sample |
Effect of parameters on antibiotic adsorption
The design of cost-effective wastewater treatment systems relies heavily on determining the equilibrium time, which is a critical parameter. In order to study the adsorption kinetics, five distinct AMX quantities were selected. Fig. 3 illustrates the relationship between the adsorption capacity (qt) of AMX onto OMWCNTs and contact time for different initial concentrations of AMX. The qt value showed a steep rise from 98.8 mg/g to 829.4 mg/g as the concentration rose from 10 mg/L to 100 mg/L, as seen in Fig. 3.
The impact of the initial concentration of AMX on the adsorption process was studied by varying it from 10 to 100 mg/L. The pH was kept constant at 7, and the amount of adsorbent was fixed at 0.2 g/L. The contact time was set at 10-150 min. According to figure 3, the efficiency of elimination reduced with the rise in the initial AMX concentration. The minimum efficiency was observed at an initial AMX concentration of 100 mg/L.
Adsorption process was also deliberated at four different temperatures to analyze its temperature dependence. The initial AMX concentrations were varied over a range, and the adsorption isotherms were used to analyze how changes in temperature affect the studied process. Fig. 4 illustrates the variation in adsorption isotherms with temperature. The experimental results indicated an enhancement in the equilibrium uptake by rising the equilibrium AMX concentrations within the experimental concentration range.
To investigate the kinetic behavior of AMX adsorption onto OMWCNTs, three commonly used kinetic models were employed, namely the PFO, PSO, IPD model. In PSO model, the rate-limiting step is the surface adsorption that involves chemisorption, where the removal from a solution is due to physicochemical interactions between the two phases 27. The model that best fit the data was designated based on the correlation coefficient values (R2) obtained through linear regression. Equation 3 represents the PFO model 28, 29.

The quantities of AMX adsorbed at equilibrium and time t are represented by qe and qt (mg/g), respectively. The rate constant of the PFO is denoted by k1 (min-1). The slope and intercept of the log(qe-qt) versus t plots can be employed to compute the values of k1 and qe. These calculations are presented in Fig 5a. The R2 gotten for all considered concentrations are in the range of 0.75-0.85 and are low. Additionally, the qe,exp (mg/g) experimental values are significantly different from the calculated qe, cal (mg/g). As a result, the PFO does not effectively describe the adsorption process.
Equation 4 expresses a PSO in a linear form 30, 31.
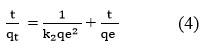
The PSO rate constant, k2 (g/mg min), can be derived from the rate equation. The values of qe and k2 can be obtained by plotting t/qt against t (Fig. 5b). The high R2 (>0.999) of the linear plots at different concentrations indicate that the adsorption of AMX onto OMWCNTs follows the PSO predominantly. Besides, the qe,cal are in good agreement with the qe, exp. Table 1 summarizes the equations related to kinetic models and the results obtained from their application.
The two aforementioned models are unable to determine the diffusion mechanism that occurs during the studied process. Therefore, the experimental data is examined using the IPD model (equation 5) 32, 33.

In above equation, kd (mg/g min1/2) signifies the IPD rate constant, and C is the intercept. The adsorption mechanism of a solute from a solution onto porous adsorbents progresses through three distinct phases. Following this, the process transitions into a stage of gradual adsorption (step II), where the rate-limiting factor becomes IPD. Finally, equilibrium is achieved, with IPD slowing down caused by the minimal concentrations of adsorbate left in the solutions 34. The regulation of the adsorption rate is influenced by one or more of these three stages.
In the graph depicted in Figure 5c, where qt is plotted against t1/2, a dual linear trend is observed. Initially, it is posited that AMX quickly migrates to the outer surface of OMWCNTs via film diffusion within a short duration. The initial linear phase likely reflects the penetration of AMX molecules into the OMWCNTs particle through IPD. Subsequently, the subsequent linear portion signifies the establishment of final equilibrium 35.
Over the past few years, the identification of human medicines and pharmaceutical drugs in sewage, wastewater, and domestic water systems has brought attention to their potential ecotoxicological effects on human health in numerous countries 36. The detrimental impacts of pharmaceuticals in water resources pose serious risks to human health, as well as to animals and plants, with far-reaching consequences 37.
The data presented in Fig. 3 demonstrate a direct relationship between equilibrium uptake and increasing concentrations of AMX within the experimental concentration range. The explanation for this could lie in the enhanced driving force resulting from the concentration gradient. For higher concentration of AMX, more AMX ions surrounded the active sites of OMWCNTs, facilitating adsorption and consequently leading to an increase in qe values 38. Additionally, it was noted that the qe rose with increasing temperature, suggesting the endothermic nature of AMX adsorption onto OMWCNTs 39, 40. It’s feasible that this arises from the strengthening of physical bonding between AMX molecules and the active sites of the adsorbent at higher temperatures, coupled with the increased solubility of AMX, which enhances interaction forces between the solvent and the solute 41.
The concentration of the pollutant (AMX) at the start of the study is a critical factor in adsorption and requires assessment. Previous research has demonstrated significant effects of this factor up to a certain limit. Additionally, the adsorption capacity of the adsorbent for AMX molecules depends on the presence of sorption sites on its surface. Once the optimal value is exceeded, the adsorption efficiency decreases due to the saturation of active sites on the adsorbent’s surface 35. Moreover, there is minimal available space between the layers of OMWCNTs, and the adsorption process involves replacing interlayer anions of the adsorbent with contaminants. This space can accommodate the ideal values of OMWCNTs and enable efficient adsorption 40. Consequently, the saturation of active sites in the interlayer of OMWCNTs prevents further diffusion of contaminants onto the adsorbent.
The concentration of AMX during initial stages significantly impacts its adsorption capacity on OMWCNTs adsorbent. The observation reveals that as the initial AMX concentration increases, the adsorption capacity of AMX also increases. A heightened driving force of the concentration gradient with the increase in the initial concentration could be the reason behind this phenomenon. During the initial stages of adsorption (contact time<45 min), the process is rapid, whereas it slows down later on. The presence of many vacant surface sites at the outset may contribute to this, making it easier for AMX molecules to adsorb. However, as the surface sites get occupied, the unoccupied sites become challenging to fill due to repulsive forces between the AMX molecules on the OMWCNTs and the bulk phase 34. The curves observed during the process are single, smooth, and continuous towards saturation, signifying that monolayer coverage of AMX molecules on OMWCNTs surface has been achieved 35.
The thermodynamic parameters provide valuable information such as the strength of adsorbate attachment to the adsorbent surface, whether the process is chemisorption or physisorption, whether it is exothermic or endothermic, and the entropic changes during adsorption. Physisorption occurs when the ∆𝐺° value is approximately 20 kJ/mol, while chemisorption occurs when the value ranges from 80-400 kJ/mol 33. According to the results of the adsorption model, the adsorption of the drug on the OMWCNTs adsorbent was determined to be physisorption. A negative ∆𝐺° value designates that the adsorption is endothermic and spontaneous. It was observed that the change in free energy decreases with increasing temperature, but the nature of adsorption is non-normal. It is possible that the reason for this is the strengthening of the adsorption process caused by rising temperature 35. The ∆𝐺° for AMX adsorption was found to be within the range of -2.2 to -8.9 kJ/mol, indicating that the adsorption was primarily physical adsorption 36. A ∆S°>0 specifies a developed randomness between solid-solution interfaces during the adsorption of AMX on OMWCNTs 38.
The graph in Figure 5c illustrates the plot between qt and t0.5 for AMX adsorption and desorption onto OMWCNTs. It is evident from the graph that both adsorption and desorption exhibit a multi-linear behavior, with two distinct steps visible in the plots representing the initial and second stages 36. The initial stage is likely associated with the boundary layer diffusion effect, while the next stage may be a result of IPD effects. The current study revealed that the rate constants (kd) governing both adsorption and desorption exhibit higher magnitudes, showing an upward trend with increasing initial AMX concentration. Consequently, the linear section at the upper portion of the graph can be interpreted as a rate parameter (kd), indicative of the adsorption and desorption rates within the domain where IPD was identified as the rate-controlling step 34.
Table 2 shows a comparison of the adsorption capacity (qe) of different materials reported in the articles as adsorbent for AMX adsorption from aqueous solution under different experimental conditions.
The mechanism of adsorption of AMX to the OMWCNTs material involves a multi-step process where molecules move into the material. This process is seen in the data as a steep part (bulk diffusion) followed by a less steep part (film and pore diffusion) (35).
Additionally, the OMWCNTs surface has different chemical features like -OH and -C=O groups that can bind to AMX in various ways, including strong (covalent) and weak (hydrogen bonding, ionic interaction, etc.) connections. The -NH2 groups can also bond with AMX through strong (imine) and weaker (amide) connections, and other interactions (Fig 6).
![]() |
Figure 3: Effect of contact time (temperature: 303 K, adsorbent dose: 0.1 g/L) |
![]() |
Figure 4: Effect of temperature (C0:100 mg/L, adsorbent dose: dose: 0.1 g/L, time: 75 min). |
![]() |
Figure 5: PFO (a); PSO (b); IPD (C) kinetic plots for AMX adsorption onto OMWCNTs. |
Table 1: Values of kinetic parameters for the adsorption of AMX
AMX (mg/L) |
(qe) exp |
IPD model |
PFO |
PSO |
||||||
Kd |
I |
R2 |
(qe) cal |
K1 |
R2 |
(qe) cal |
K2 |
R2 |
||
10 |
41.55 |
2.11 |
11.62 |
0.752 |
11.6 |
0.451 |
0.895 |
21.2 |
0.004 |
0.997 |
25 |
81.34 |
3.65 |
25.47 |
0.787 |
19.47 |
0.274 |
0.912 |
65.2 |
0.001 |
0.996 |
50 |
119.1 |
7.94 |
29.76 |
0.884 |
28.94 |
0.011 |
0.904 |
161.2 |
0.0009 |
0.994 |
75 |
155.1 |
10.25 |
38.48 |
0.816 |
59.73 |
0.046 |
0.876 |
214.2 |
0.0004 |
0.998 |
100 |
196.1 |
14.2 |
44.3 |
0.793 |
67.2 |
0.074 |
0.871 |
251.7 |
0.0007 |
0.992 |
![]() |
Figure 6: Adsorption mechanism of AMX onto OMWCNTs |
Table 2: Comparison of the maximum adsorption of various adsorbent for AMX
Adsorbents |
Qe (mg/g) |
Ref |
AC- Jujube nuts |
56.2 |
13 |
MWCNT |
465.1 |
14 |
AC- cashew of Para |
56.95 |
15 |
AC-Date Pits |
84.93 |
18 |
AC-olive stone |
84.1 |
21 |
Synthesized NiO |
94.3 |
26 |
Magnetic graphene oxide |
93.2 |
34 |
OMWCNTs |
926.2 |
This study |
Conclusion
In the present investigation, it was found that the adsorbent demonstrated a maximum uptake capacity of 829.4 mg/g and achieved a total percentage removal of 98.71% of AMX from the aqueous solution. Optimal physicochemical parameters were determined to be a dosage of 0.1 g, a contact time of 75 minutes, and a temperature of 40 °C, respectively. Various adsorption kinetic models, including the PFO, PSO, and IPD models, were employed to assess the kinetics of maltose sorption by OMWCNTs. The results indicate that the sorption of AMX onto OMWCNTs conforms well to the PSO.
Acknowledgement
The authors are grateful to Zahedan University of Medical Sciences, because of supporting this research (IR.ZAUMS.REC.1401.147).
Funding Sources
The author(s) received no financial support for the research, authorship, and/or publication of this article.
Conflict of Interest
The author(s) do not have any conflict of interest.
Data Availability Statement
This statement does not apply to this article.
Ethics Statement
This research did not involve human participants, animal subjects, or any material that requires ethical approval.
References
- Fei Y, Li Y, Han S, Ma J, J. Colloid Interface Sci. 2016; 484, 196–204.
CrossRef - Balarak D, Mahvi AH, Shim MJ, Lee SM. Desalin Water Treat. 2021; 212, 390-400.
CrossRef - Ahmadi S, Banach A, Mostafapour FK. Desalin Water Treat. 2017; 89: 297-303.
CrossRef - Yilmaz M, Al-Musawi TJ, Saloot MK. Biomass Conv. Bioref. 2022. https://doi.org/ 10.1007/s13399-021-02279-y
- Al-Musawi TJ, Mengelizadeh N, Taghavi M. Biomass Conv. Bioref. 2021. https://doi.org/10.1007/s13399-021-01962.
- Wang H, Fang C, Wang Q, Chu Y, Song Y, Chen Y, Xue X. RSC Adv. 2018; 8:16260–16268.
CrossRef - Wang J, Zhang M, Zhou R, Li J, Zhao W, Zhou J. Water Sci Technol. 2020; 82:242–254.
CrossRef - Wu S, Hu YH. Chem Eng J. 2021; 409:127739.
CrossRef - Mahvi AH, Mostafapour FK. Fresenius Environ Bull. 2018; 27(8):5759-67.
- Balarak D, Taheri Z, Shim MJ, Lee SM, Jeon C. Desalin Water Treat. 2021; 215; 183-193.
CrossRef - Azarpira H, Mahdavi Y, Khaleghi O. Pharm Lett. 2016; 8(11):107-13.
- Shikuku VO, Zanella, Kowenje CO. Appl Water Sci. 2018; 8, 175. https://doi.org/ 10.1007/s13201-018-0825-4.
CrossRef - Belaissa, Y., Saib, F. & Trari, M. Reac Kinet Mech Cat; 2022; 135, 1011–1030.
CrossRef - Balarak D, Mostafapour FK, Bazrafshan E, Saleh T. A. Water Sci Technol. 2017; 75; 1599-1606.
CrossRef - Lima, DR., Lima, EC., Umpierres, C.S. Environ Sci Pollut Res. 2019; 26, 16396–16408. https://doi.org/10.1007/s11356-019-04994-6.
CrossRef - Leonard JM. Githinji, MKM., Ramble O. Ankumah. Water Air Soil Pollutant, 2011. 2019: 191-201.
CrossRef - Ibáneza M, Bijlsmaa L, Moralesb E, Pastorb L, Hernándeza F. J. Hazard. Mater. 2013. 260: 389-398.
- Belhachemi, M., Djelaila, S. Environ. Process. 2017; 4, 549–561.
CrossRef - Boukhelkhal, A., Benkortbi, O., Hamadeche, M. Water Air Soil Pollut. 2015; 226, 323. https://doi.org/10.1007/s11270-015-2587-z.
CrossRef - Moarefian, A., Golestani, H.A. & Bahmanpour, H. J Environ Health Sci Eng. 2014; 12, 127. https://doi.org/10.1186/s40201-014-0127-1.
CrossRef - Limousy, L., Ghouma, I., Ouederni, A. Environ Sci Pollut Res. 2017; 24, 9993–10004. https://doi.org/10.1007/s11356-016-7404-8.
CrossRef - Gao H, Zhao S, Cheng X, Wang X, Zheng L. Chem. Eng. J. 2013. 223; 84–90.
CrossRef - Balarak D, Mostafapour FK, Joghataei A. Pharm. Chem. 2016, 8(8):138-145.
- Balarak D, Mahdavi Y, Bazrafshan E, Mahvi AH, Esfandyari Y. Fluoride. 2016; 49(1)71-83.
- Naghsh N, Chandrika K, Balarak D. Int. J. Pharm. Investigation. 2024;14(2):365-70.
- Eltaweil AS, Abd El-Monaem EM., El-Subruiti GM. J Porous Mater. 2023; 30, 607–618.
CrossRef - Balarak D, Chandrika K. Int J Pharm Investig. 2019;9(3):117-21.
CrossRef - Rout, DR, Jena, HM. Environ Sci Pollut Res. 2022; 29, 32105–32119. https://doi.org/10.1007/s11356-021-17944-y.
CrossRef - Mostafapour FK, Bazi M, Siddiqui SH, Bagheri H. Int J Pharm Investig. 2021;11(4):384-8.
CrossRef - El Rouby WMA, Farghali AA, Sadek MA. J Inorg Organomet Polym. 2018; 28, 2336–2349. https://doi.org/10.1007/s10904-018-0885-9.
CrossRef - Li R, Wang Z, Zhao X. Environ Sci Pollut Res. 2018; 25, 31136–31148. https://doi.org/10.1007/s11356-018-3064-1.
CrossRef - Balarak D, Ganji F, Chandrika K, Haseeb S. Int J Pharm Investig. 2020;10(2):122-6.
CrossRef - Mostafapour FK, Haseeb S, Balarak D, Moein H, Sajadi AA, Jalalzaei Z. Int J Pharm Investig. 2021;11(1):41-5.
CrossRef - Kong X, Liu Y, Pi J. Environ Sci Pollut Res. 2017; 24, 6679–6687.
CrossRef - Liang, H., Zhu, C., Ji, S. Biochar. 2022; 4, 3. https://doi.org/10.1007/ s42773-021-00130-1.
CrossRef - Shan DN, Deng SB, Zhao TN, Wang B, Wang YJ, Huang J, Yu G, Winglee J, Wiesner MR. J Hazard Mater. 2016; 305:156–163.
- Zisti F, Chandrika K. Int. J. Pharm. Investigation. 2023;13(4):778-83.
CrossRef - Azadfar M, Tahermansouri H, Qomi M. J. Chin. Chem. Soc, 2021;68:2103–2117.
CrossRef - Gholizadeh, H, Ghorbani-HasanSaraei A, Tahermansouri H. Carbon Lett. 2019; 29, 273–279.
CrossRef - Gholizadeh, H,Ghorbani-HasanSaraei A, Tahermansouri H. Shahidi SA. J. Chin. Chem. Soc, 2020;67: 546–557.
CrossRef
This work is licensed under a Creative Commons Attribution 4.0 International License.